Going from Gauzy to Granular
Anna Devor and collaborators aim to extract neuronal circuit activity from fMRI, opening door for clinical applications
By Patrick L. Kennedy
Say you’re listening to a lecture and paying close attention. When that happens, your brain releases a chemical called acetylcholine. Later, though, you start to doze off. Suddenly, the professor calls your name. You open your eyes and attempt to orient yourself. That means your brain has released a chemical called norepinephrine. Finally, after class, you head to the gym for a good workout, releasing two other chemicals, dopamine and serotonin.
All of these chemicals are known as neuromodulators. They orchestrate our brain rhythms—the patterns of neuronal circuit activity that vary in space and time across different behaviors, arousal level and sleep states. But just as a computer circuit is broken when a component goes missing, says Associate Professor Anna Devor (BME), a neuronal circuit malfunctions when it loses a neuromodulator.
The result can be mental illness. “Something is wrong with the way information is being processed,” says Devor.
The neuromodulator acetylcholine has been implicated in neurodegenerative diseases such as Alzheimer’s and some types of dementia. “Acetylcholine is a chemical that helps coordinate activity across brain regions,” says Devor. A shortage of it is “one of the first things that happens in people with Alzheimer’s.” And yet, she adds, “There’s no test for it.”
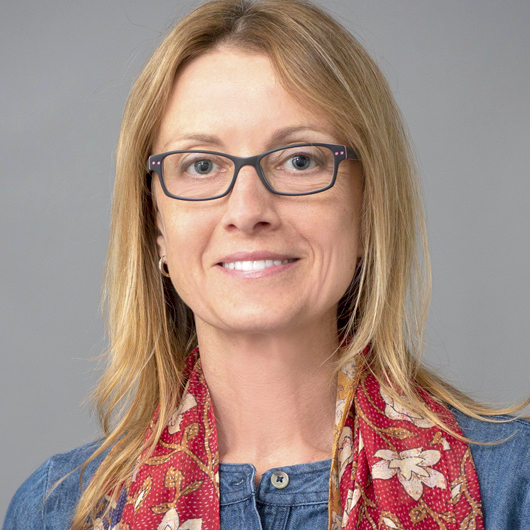
But Devor and a team she leads have an idea for how a technology that already exists will someday be used to pick up on warning signs, such as reduced or dysfunctional neuromodulation. The Boston University team, which includes associate professors Laura Lewis and Michael Economo (BME) and Lei Tian (ECE, BME), as well as colleagues at UC San Diego, MGH and UIC, have received a large collaborative NIH BRAIN Initiative award to develop a method for extracting information about neuronal circuit activity from functional magnetic resonance imaging (fMRI) scans. With the NIH grant, the team will conduct a multi-year, multi-institution effort combining parallel human and animal studies, as well as computational modeling.
“The point is to be able to take noninvasive imaging,” says Devor, referring to fMRI scans, “and infer something about the underlying neuronal circuits, which currently is not possible.”
fMRI is used to map fast brain activity by measuring the relatively slow flow of blood to its different regions. Researchers can look at synchronicity in the fluctuations of fMRI signals across brain regions, but “this is kind of where the analysis ends,” Devor says. The underlying picture of what’s happening with the neurons and circuits remains blurry.
However, blood flow is linked to—indeed, driven by—neurons. As neurons pulse, the cerebral arteries dilate. That rhythmic activity is slow enough to be detected with fMRI. Devor and collaborators seek a quantitative understanding of the relationship between brain rhythms, the release of neuromodulators, and the resulting fluctuations in fMRI signals across the brain. Their hope is to make that blurry picture of blood flow resolve into a crystal-clear image of neuronal circuits.

How? Thankfully, researchers can see neurons, neuromodulators and blood vessels in action, in fine detail, in model organisms such as mice using in vivo optical microscopy. The team will study mice while they’re performing tasks that require concentration, triggering the release of acetylcholine. “With optical technologies, we can get very high resolution and specificity,” Devor says. “We can see what types of neurons talk to what other types of neurons—we can see the circuit.”
Then, similar studies will be conducted with mice but using fMRI instead. “This gives us an important stepping stone to get from the microscopic measurements in mice to something that can be directly translated to humans, because now we’re comparing fMRI to fMRI,” says Devor.
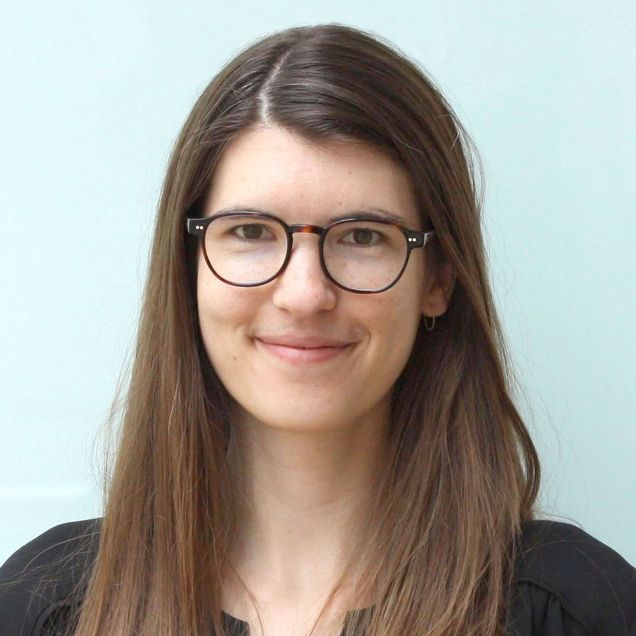
In parallel, the team will conduct fMRI studies with human volunteers performing cognitive tasks. “We plan to use these fMRI signals to predict what type of brain state a person is in—for example, decoding whether they are attentive or distracted, or have high or low norepinephrine,” says Lewis, who will be conducting these studies in collaboration with colleagues at MGH. By providing a link across species, the human fMRI studies will ultimately allow noninvasive measurement of these important aspects of brain state and neuromodulatory chemicals in humans.
Eventually, Devor and Lewis hope, that stepping stone will give clinicians a kind of calculus they can use to fill in the blanks—a way to deduce granular, cellular-level, circuit-specific data from the blood-flow-level fMRI results of any human patient.
“We’d like to put a person in the fMRI scanner and be able to say whether or not this person has healthy acetylcholine activity,” Devor says. “If we can make this difference, it’s going to be very important in terms of sorting patients early on—who is likely to go which way?”