Brains and Seahorses in Pursuit of Memory
Memories thread and unify our sense of being. How can our brains achieve such a feat as remembering?
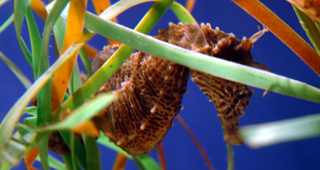
The hippocampus, named after the Greek for seahorse, is what makes our memory work.
The brain is a remarkably complex jungle of neurons. Its underlying neurobiological computations have made possible the whole of human civilization – from the stone tools of Homo habilis to that ineffable phenomenon we call culture. Every day, neuroscientists take on the tall order of revealing the neural principles that govern the cognitive processes underlying our representation of the world around us. At Boston University’s Laboratory of Cognitive Neurobiology, headed by Dr. Howard Eichenbaum, we approach the problem by studying the neural mechanisms that give rise to memory. In particular, we utilize electrophysiological and neuropsychological techniques to investigate how information is represented by the hippocampus and other related brain areas nestled deep within the brain. In our latest project, we are investigating how episodic memory, the memory of personal experiences,1 is represented by neurons in the rodent hippocampus.2
Remember Me?
Imagine that you are walking to class. As you walk past a group of students, one of them yells “Hi,” but – oops – you forget how you know this person. After a few seconds of hesitation, you nod back and continue to walk. Later, something clicks and you suddenly remember that you were introduced to the student last weekend at a friend’s party. What’s more, this memory is accompanied by a rich representation of your meeting. You might remember how the conversation unfolded-you first talked about which classes each of you are taking, then you both lamented about your housing situation, then thirst struck, and you both made a beeline to the drinks table for a refill. Up until this moment, you were only familiar with the person but could not recollect the details of where and when you had met. Familiarity describes what somebody feels when they know that they’ve met the person somewhere before, but cannot remember the contextual details of their meeting. On the other hand, recollection is the process by which the memory of a certain event (what), its place in time (when), and position in space (where) is consciously accessed.3 In particular, our ability to accurately recall the temporal order of the events interwoven into a memory is a hallmark of episodic memory and fundamentally depends on the hippocampus.4 We’ve all had these moments, but what is it about our brains that allows us to recollect some memories so vividly while others remain fuzzy and distant?
Seahorses and Memory
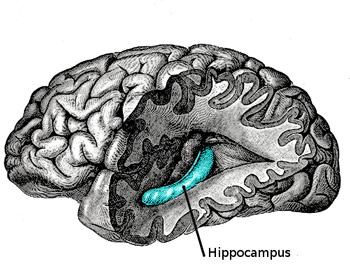
The hippocampus is a bilateral structure tucked inwardly in the medial temporal lobe. It is a neural structure of considerable research given its proposed role in memory formation. Lesions to the hippocampus cause profound memory impairments as observed in amnesiac patients.
The term “hippocampus” is derived from the Greek word for “seahorse,” (hippos meaning horse, and kampos meaning sea monster) in light of its curved, tube-like structure. Before we can understand its function, we must briefly tour the structural circuitry of the hippocampal memory system, of which the hippocampus is only a component. Interestingly, the hippocampus is one of the most phylogenetically conserved neural regions in mammals in terms of structure and function, which allows for relevant animal studies on our evolutionary cousins – the rat.5 The hippocampus is a bilateral structure which, in humans and non-human primates, lies slightly above the ears and is tucked inwardly in the medial temporal lobe. The flow of information within the hippocampus and adjacent areas usually is depicted as a loop: entorhinal cortex (EC) → dentate gyrus → CA3 → CA1 → subiculum → entorhinal cortex. The connection from EC to CA1 involves three synapses – specialized junctions through which neurons communicate – and this circuit is often referred to as the trisynaptic loop.6 The entorhinal cortex itself receives inputs from various nearby regions, such as the perirhinal and parahippocampal cortex, which in turn receive input from various neocortical areas (e.g., from frontal lobe, parietal lobe, temporal lobe) and thus provides a bridge between the hippocampal network and the rest of the brain.
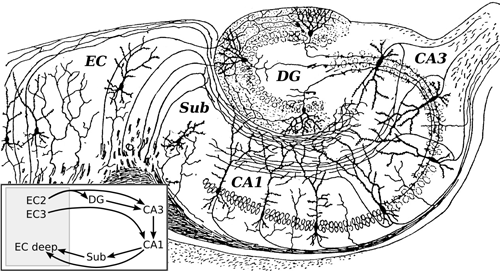
The father of neuroscience, Santiago Ramón y Cajal, pioneered microscopic investigations of the brain’s neural circuitry, such as the rodent hippocampus. The hippocampus can be subdivided into multiple regions, such as the entorhinal cortex (EC), subiculum (SUB), CA1, CA3, and dentate gyrus (DG).
Neuroscientists spend a considerable amount of time researching how information is processed in the hippocampus in hopes of revealing how this information changes as it progresses through the trisynaptic loop. One approach to answering this question is to ask how each sub-region uniquely contributes to aspects of episodic memory. Two recent experiments that speak to this approach involve training rats on a paired associate task.7,8 With human subjects, paired associate tasks typically involve learning arbitrary associations between word-pairs, such as flower-dog. Human amnesics are profoundly impaired when presented later with only one of the words in a pair (e.g., flower) and are asked to recall its associate (e.g., dog).9,10
Accordingly, hungry rats were trained to associate one object (A) with one odor (A’) and a second object (B) with a second odor (B’).11 During a single trial in the experiment, one of the objects (A) is presented for a few seconds to allow for its vigorous exploration by the rat. The object is taken away and, after ten seconds, a container filled with sand scented with the odor associate (A’) is presented to the rat. The hungry rat digs in the sand to retrieve a food reward buried in the bottom of the sand. The same sequence of events takes place during a subsequent trial that uses the other paired associate (B-B’). During training, however, rats were also given trials in which there was a mispairing between the object and odor such as A-B’ or B-A’. During these trials, the sand is not baited with food reward and the rat is to refrain from digging.
After exposure to the several types of trials, normal rats learn to dig in the sand to retrieve a reward when the odor was paired with its proper object, and refrain from digging when the trial was a mismatch between the object and odor. Interestingly, while normal rats learned this relationship, rats with bilateral lesions of CA1 showed no evidence of learning. More striking was the observation that rats with bilateral lesions of CA3, which lies immediately adjacent to CA1 within the hippocampus, did learn this relationship.
In an earlier experiment, the authors found that bilateral lesions of CA1 do not impair paired associate learning in a different variation of the paired associate task.12 Importantly, in this variation, the paired associates are presented simultaneously rather than sequentially so that the rats do not have to endure a ten-second delay to complete the paired associate sequence. In this experiment, however, the authors found that rats could not learn this task if their CA3, rather than CA1 was bilaterally lesioned. Bilateral lesions of CA1 had no effect on rats learning this task.
These results emphasize the difference between the information processed by the two brain regions. More specifically, the CA1 region appears to be particularly important when the memory requires accurately recalling a sequence of events separated in time.
What’s the Purpose of the Hippocampus?
It has been proposed that the hippocampus mediates the processing of sequential and context-specific information about events that constitute episodic memories. Indeed, the hippocampus has the capacity to identify and bind common features of different episodes separated in time. Such linked episodic representations can support inferences from memory; i.e., information acquired between distinct experiences can be connected somewhat arbitrarily to form new associations between memories.4,11 After remembering where you met a friend of yours, this may be why you soon afterwards also remembered who else went to the party, how the night ended, what classes you had to miss the next day, and so on.
“No brain region acts in isolation, and the formation of an episodic memory recruits the brain’s faculties to the fullest.
Still, a caveat is worth reiterating-the hippocampus itself is only one component of a large memory system composed of several interacting brain regions. No brain region acts in isolation, and the formation of an episodic memory recruits the brain’s faculties to the fullest, engaging the full expanse of the brain from neurons to interacting networks distributed throughout high-level sensory areas, including vision, audition, olfaction, somatosensation, and even motor areas. While the information encoded in an episodic memory is initially stored in the hippocampus, there is some evidence to suggest that over time, some elements from the memory are “relocated” to the neocortex.13 Therefore, while the hippocampus plays a crucial role in recalling an episodic memory for a particular event, it may do so by facilitating an interaction among neocortical, parahippocampal, and hippocampal structures to effectively bind all of the essential elements from an episode together.3
Electrophysiological Approaches to Studying Episodic Memory
The brain speaks in electricity – it is the means by which neurons represent information. Electrophysiology is the study of electric phenomena and its relation to biological processes. In cognitive neurobiology, electrophysiology is often utilized to probe either the activity of single neurons or large populations of neurons. This is possible because of a neuron’s intrinsic electrical properties. When a neuron fires, or spikes, it creates a local difference in electrical potential, and the summed activity of many active neurons creates what is known as an extracellular field potential. This intrinsic property of active neurons allows us to investigate hippocampal activity by recording the field potentials of various neurons firing simultaneously during the acquisition of an episodic memory.
Hyperdriving at the Speed of Neurons
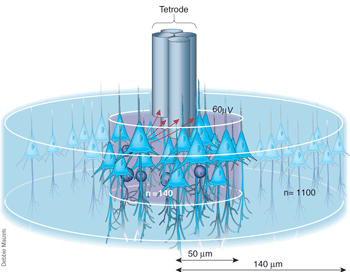
A tetrode consists of four insulated wires that are twisted and heated in order to optimize their capacity to conduct electricity. Once implanted in a brain, tetrodes permit accurate detection of individual neurons. Image courtesy of Nature.
A fundamental advantage of electrophysiological techniques is that they allow us to probe neural activity in vivo while the animal performs any particular task. Often, devices called hyperdrives are implanted in rats to record neuronal activity in vivo. Hyperdrives contain multiple wires. Each wire, or tetrode, actually consists of four insulated wires that are twisted and heated in order to optimize their capacity to conduct electricity. Hyperdrives are divided into numerous drivers, which act as distinct sections that allow for independent manipulation along a vertical axis of a single tetrode.
Following surgical implantation, each tetrode is lowered individually into the rat brain until it reaches the point of interest. In this case, the point of interest is the CA1 region of the hippocampus. The use of two or more electrodes allows for the triangulation of distances between a neuron and the electrodes via the recorded amplitude of the neuron firing.14 In other words, having four wires wrapped around each other permits accurate detection of individual neurons such that the degree of the local potential difference recorded at the tip of each individual wire in the tetrode has a slightly different magnitude. This property has allowed computer scientists to create algorithms that sort and detect the activity of individual neurons from hundreds of neural signals sent to each tetrode, a technique called spike sorting.
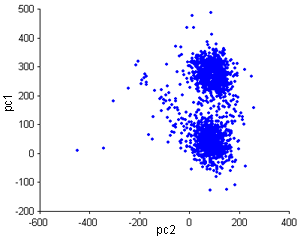
Spike sorting is a technique used in the analysis of electrophysiological data. It is the process of separating and assigning spikes to different neurons, two of which are displayed. Spike sorting algorithms use the information from electrodes in the brain to distinguish the activity of one or more neurons from background electrical noise.
Thus, tetrodes can record extracellular electrical activity and transmit the signal to an electrical board, which in turn sends the signal to a computer for visualization and analysis. This makes it possible to monitor neural activity during a variety of tasks.
Current Research from the Eichenbaum Lab
At the Eichenbaum Lab, we utilize electrophysiological techniques in order to study neural activity and distinct firing patterns in the rat hippocampus during the memory formation of a sequentially ordered and recently experienced event (i.e. an episodic memory).
The Project and Predictions
As discussed above, the CA1 sub-region is believed to be integral to processing sequential information between items. However, the neuronal mechanisms by which memories are created and accessed in this manner are poorly understood and are the subject of considerable debate. It is also well known that CA1 neurons can encode both, head movement and the position of the rat in its environment.15
In order to better isolate any potential neural activity that is influenced by processing sequential information, we designed a paired associate task that makes use of a head-restraining apparatus. One advantage of using a head-restrained preparation is that we eliminate environmental or body-derived inputs while recording data in vivo from a rat’s hippocampus. Thus, our recordings of CA1 activity avoid confounding variables and might be strongly linked with representing sequential information between items.
In our task, which is conceptually similar to the experiments discussed above, the restrained rat will learn to pair an odor with a specific auditory cue associate across a ten second delay. In our version, rather than using objects for investigation, we will use two auditory cues (sounds A and B) that can be paired or mispaired with two possible odor cues (odors 1 and 2) that are puffed directly at the rat’s nose after a 10 second delay. For two of these auditory cue/odor combinations (paired associate trials: A-1 and B-2), the food-deprived rat will learn to lick at a lickspout next to its mouth to receive a sugar-water reward. For the mispair trials (A-2 and B-1), it will learn to refrain from licking. In order for the rat to perform well on this task, it must accurately process and recall the sound-odor sequence to decide whether to lick or not. The experiment consists of an odor (what), the place in which it was experienced (where), and the order in which the presentations occurred (when), thus capturing the essential components of an episodic memory.16
Each rat will be surgically implanted with a hyperdrive, which will feed 24 tetrodes into the CA1 region of the hippocampus in order to monitor the extracellular electrical potential of a population of neurons. Each neuron’s activity will be assessed and compared for firing discrepancies during various points of the trial (i.e. stimulus presentation, trace-interval, and odor sniffing). We hypothesize that CA1 neurons will respond distinctly to separate events that make up the trial, but the particular event that reliably evokes a response will vary across the neuronal population. We predict that CA1 neurons will become more active during the delay period between the presentation of an auditory cue and subsequent odor, thus acting as a network to encode the sequential structure of an episodic memory.
Maybe You Forgot Me?
When all is said and done, – and indeed, more is often said than done – our goal is to apply all this neuroscience to humans. Pop quiz: What will certainly kill us all? (Hint: Hamlet referred to it as the “Great Equalizer”). Good answers include poverty, cardiovascular disease, and strokes, but they are all wrong. They lack a single component: inevitability. It has been keenly observed that aging is the number one cause of death in the world. From the womb to the cradle, the schoolyard to the cubicle, we are all configured to experience the full circle of life.
“Understanding episodic memory will allow us to predict symptoms and point to possible treatments when its activity wanes.
Our hippocampal neural system makes all of life’s boons memorable – memories of our first kiss, graduating college, marriage – but it is far from perfect. Since aging comes with the accumulation of small neural errors in the form of cell damage or death, what happens when aging forces our neural machinery to break down? Alzheimer’s disease (AD) is just one example of an age-related neuropathology that can be better understood in light of the aforementioned studies. It is a neurodegenerative disease affecting nearly 27 million people worldwide17 and is the most common form of age-related dementia.18
Among the first areas targeted by AD is the hippocampus, giving rise to characteristic memory deficiencies and reduction in cognitive abilities. Identifying the neural underpinnings behind memory formation may yield important developments in the treatment of AD patients, in whom episodic memory is profoundly impaired.19,20
This research raises several inevitable questions: how will a person with AD be affected in terms of episodic memory? Will he or she have trouble interpreting the context of a situation or past memory? What about the temporal order of events? How do the data regarding animal models with hippocampal lesions compare to humans in whom the hippocampus has deteriorated? Our hope is to reveal the hippocampus’s role in episodic memory formation in order to understand how this brain region functions effectively and interactively, which will allow us to predict symptoms and point to possible treatments when its activity wanes.
Such is the power of neuroscience, and for us at the Eichenbaum lab, scientific progress manifests itself while studying brains and seahorses in the pursuit of memory.
References
1Tulving, E. 1983. Elements of Episodic Memory. New York: Oxford University Press.
2Eichenbaum, H. 2004. Hippocampus: Cognitive processes and neural representations that underlie declarative memory. Neuron. 44:109-120.
3Eichenbaum H, Yonelinas AP, Ranganath C. The medial temporal lobe and recognition memory. Annu Rev Neurosci. 2007;30:123-52.
4Fortin, N.J., Agster, K.L., and H.B. Eichenbaum. 2002. Critical role of the hippocampus in memory for sequences of events. Nature Neuroscience. 55:458-62.
5Manns, J.R., and H. Eichenbaum. 2006. Evolution of declarative memory. Hippocampus. 16:795-808.
6Amaral, D.G. & Witter, M.P. 1989. The three-dimensional organization of the hippocampal formation: a review of anatomical data. Neuroscience. 31, 571-591
7Bunsey M, Eichenbaum H. 1993. Critical role of the parahippocampal region for paired-associate learning in rats Behav Neurosci. Oct; 107(5):740-7.
8Bunsey M, Eichenbaum H. 1996. Conservation of hippocampal memory function in rats and humans. Nature. Jan 18;379(6562):255-7.
9Shimamura, A. P., & Squire, L. R. 1984. Paired associate learning and priming effects in amnesia: A neuropsychological study. Journal of Experimental Psychology: General, 113, 556-570.
10Winocur, G., & Weiskrantz, L. 1976. An investigation of paired-associate learning in amnesic patients. Neuropsychologia. 14, 97-109.
11Kesner, R.P., Gilbert, P.E., and M.R. Hunsaker. 2005. The role of CA1 in the acquisition of an object-trace-odor paired associate task. Behav Neurosci. 119:781-6.
12Lee I, Hunsaker MR, Kesner RP.The role of hippocampal subregions in detecting spatial novelty. 2005. Behav Neurosci. Feb; 119(1):145-53.
13Ji D, Wilson MA. 2007. Coordinated memory replay in the visual cortex and hippocampus during sleep. Nat Neurosci. Jan;10(1):100-7. Epub Dec 17.
14Buszaki, G. et al. 2008. Internally generated self-assembly sequences in the rat hippocampus. Science. 321:1322-1327.
15Taube JS, Muller RU, Ranck BJ Jr. 1990. Head-direction cells recorded from the postsubiculum in freely moving rats. I. description and quantitative analysis. J Neurosci 10:420-435.
16Ergorul C, Eichenbaum. 2004. The hippocampus for “what,” “where,” and “when.” Learn Mem. July; 11(4): 397-405
17Brookmeyer et al. 2007. Forecasting the global burden of Alzheimer’s disease. Alzheimer’s and Dementia. 3 (3):186-91.
18Mölsä PK, Marttila RJ, Rinne UK. 1986. Survival and cause of death in Alzheimer’s disease and multi-infarct dementia. Acta Neurol. Scand. 74 (2): 103-7
19Carlesimo, G.A., and M. Oscar-Berman. 1992. Memory deficits in Alzheimer’s patients: a comprehensive review. Neuropsychology Revews. 32:119-69.
20Fleischman, D.A., and J. Gabrieli. 1999. Long term memory in Alzheimer disease. Current Opinion in Neurobiology. 9:240-244.