All in the Timing
Killing off cancer before doing too much damage to the cells
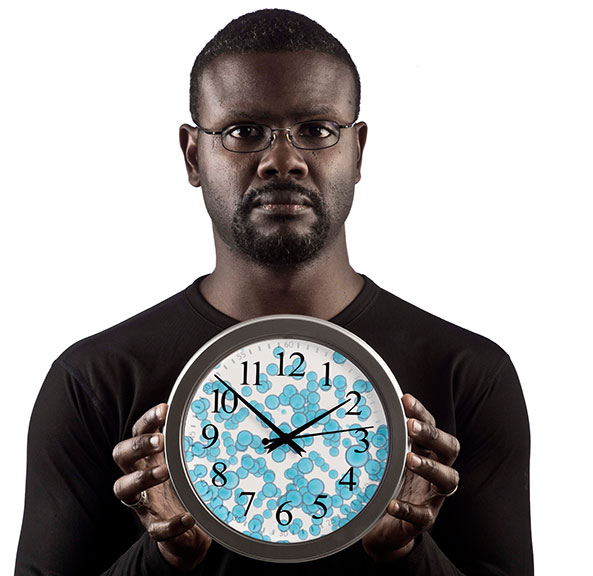
In the battle against cancer, the collateral damage can be devastating. Chemotherapy is one of the biggest weapons in the anticancer arsenal, but while the treatment kills tumors, it can poison healthy tissue, too.
According to the American Cancer Society, more than 580,000 Americans were expected to die from cancer in 2013. Over the same period, about 1.6 million people were newly diagnosed with the disease. For the majority, their treatment will include multiple injections of one or more chemotherapy drugs. Chemo’s familiar side effects are bad enough: nausea, diarrhea, fatigue, and hair loss. But there can be more severe effects, too, including nerve damage, a crippled immune system, heart toxicity, and kidney failure.
“It’s a race. You want to kill off the cancer before doing too much damage to the healthy cells,” says Tyrone Porter, associate professor of mechanical engineering at Boston University’s College of Engineering.
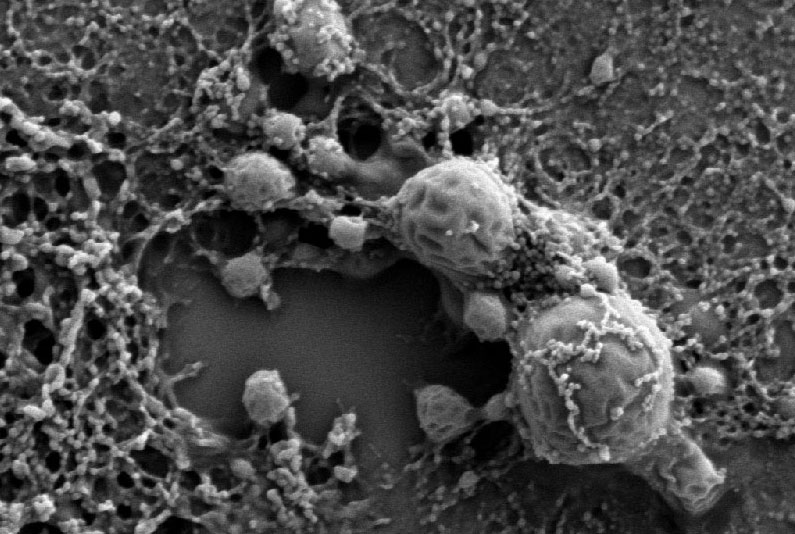
Supported by several grants, including a four-year, $1.8 million award from the National Institutes of Health, Porter wants to ensure that a patient’s healthy tissue wins that race. His lab designs tiny particles—twenty times smaller than red blood cells—that can carry chemotherapy directly into tumors to deliver their drug payload.
Porter founded the Nanomedicine & Medical Acoustics Laboratory in 2006 to focus on engineering precisely targeted chemotherapy, as well as to devise nanomedicine therapies for other killer diseases, such as heart disease. His clinical collaborators include David Seldin, chief of hematology-oncology at Boston Medical Center and a professor of medicine at the School of Medicine, and Nathan McDannold, research director of Brigham and Women’s Hospital, a teaching affiliate of Harvard Medical School. They’ve successfully tested nanodrug delivery in tissue-mimicking hydrogels, cultured cells, and animal models. We recently sat down with Porter to discuss the big promise of small medicine.
BU Research: What led you to cancer research?
Tyrone Porter: When I was in high school, one of my parents’ dear friends succumbed to throat cancer. She was in her forties, and we watched her endure the common effects and toxicities of chemotherapy, along with other treatment options. None of it worked. It was the first time I’d seen my parents deal with the death of somebody who was really close to them.
I saw the impact of the disease on people’s lives, the pain and the grief, and I started to think about how could I get involved in improving cancer treatment. When I finally had the chance to lead my own lab, that was one of the things that had always been in the back of my mind. There’s got to be a much more effective way of treating cancer patients.
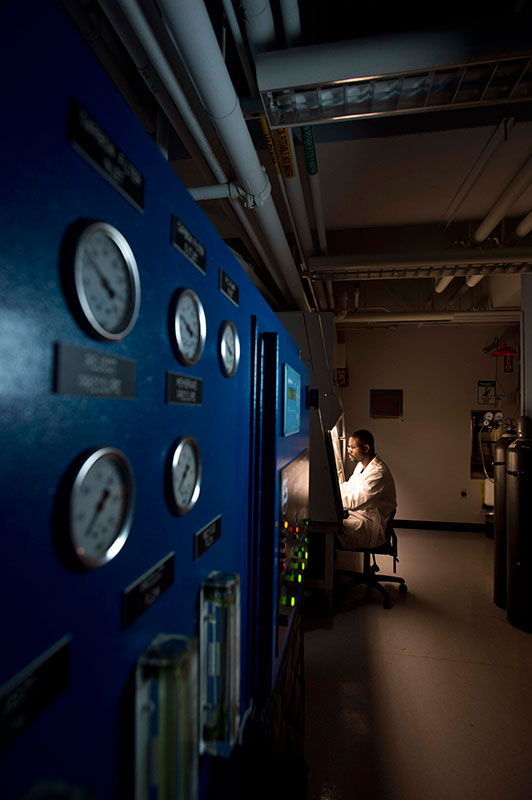
How can nanotechnology help?
As cancer grows, it needs to stimulate an increase in new blood vessel growth, and it has to be rapid, because these cells are growing much faster than normal cells. As a result, the blood vessels don’t grow the same way that they would grow in healthy tissue. They don’t seal properly. There are little defects and openings along the walls, and submicron particles can penetrate those defects. So we’re designing nanoscale particles to take full advantage of those defects in the vasculature of cancer.
We are designing drug-loaded nanoscale particles that accumulate in the tumors over time, and then degrade slowly, or in response to a stimulus, and release their chemo drug directly into the tumor. It’s very comparable to cold medicine with a 24-hour slow-release capsule to get relief from your symptoms for a full day. They put the cold medicine into a larger capsule that stomach acids break down slowly. It’s a similar idea.
How do you make these tiny particles?
We make different types, but they all pretty much go through the same process. We will either use polymers—a sort of plastic—or we use biological material called phospholipids, which are a main component of cells.
We have to dissolve these materials in solutions that don’t mix very well, so we agitate them mechanically. It’s almost like a salad dressing of oil, water, vinegar, and some seasonings. If you don’t shake it up, you’re going to get separation. But if you shake it up and everything disperses, you get little droplets of oil separating from the vinegar, because they don’t mix well.
It’s a very similar process. If you shake up these polymers, or the phospholipids, they naturally form tiny particles. The trick is to shake them appropriately, so the submicron particles will form. Then we just have to coat them so they don’t fuse and form larger particles.
Once these particles accumulate in a tumor, what triggers the drug release?
My lab has gone through sort of an evolutionary process on how to do this. You can design these carriers to release their drug in response to a variety of different stimuli. We were initially looking at designing temperature-sensitive particles that become leaky when you raise the temperature locally.
How do you heat a tumor?
We used ultrasound. While most people think about ultrasound as a diagnostic tool—to get an image of a developing fetus in the womb, for instance—I’m talking about a more high-powered ultrasound used for therapeutic applications. It’s a focused beam that, with millimeter precision, can actually heat tissue by a few degrees, up to tens of degrees.
When the temperature of the tumor is heated by three and four degrees above the body temperature, it triggers drug release on the spot. It’s like on-demand, on-the-spot chemo infusion. That was the original idea.
Did it work?
Yes, it works. We can apply ultrasound to heat where and when we want to and trigger drug release in tens of minutes. So we accomplished on-the-spot and on-demand drug infusion, and the tumors shrank. But the approach is limited to local triggering of drug release, and many cancer patients actually succumb to metastatic cancer. The cancer spreads everywhere, and you’d have to pinpoint all these targets for ultrasound heating. It isn’t ideal.
What did you try next?
Well, these particles can be biodegradable, so they just degrade over time and naturally fall apart. Or there are enzymes that the cancers release to help break down the micro-environment around them so they can invade neighboring tissue. We can take advantage of those enzymes to degrade these nanoparticles as well.
But we’ve also tried to target the particles so they’re internalized by the cancer cells and wrapped into their vesicles. When that happens, the cells try to identify the particle, and if it’s foreign to them, they try to break it down with acid. The particles we’re designing respond to that and release their drug inside the cells. So, we’ve moved from an extracellular chemo drug release via an externally applied trigger to an extracellular release using the enzymes that the cancer cells produce to an internalization of the targeted particle and drug release through acidification.
How do you know when enough of these particles have made their way in a tumor?
We started labeling them by incorporating a magnetic resonance (MR) contrast material, because magnetic resonance imaging is available in most hospitals.
How long does the process usually take?
It takes about two hours to see a plateau in the intensity of the MR contrast material in the cancer, and so we think we saturate the cancer in a couple of hours. These particles can remain stable for a few more hours, and then the body clears them out. So, there’s a window of between two and eight hours, and that’s the sweet spot.
The idea of nanomedicine has been around for a few decades, but there aren’t that many nanoparticle drug delivery systems on the market. The biggest barriers have been achieving a stable particle and getting the time-release right. If you just put these particles in water, they’re very stable. But once you put them in blood plasma, there are a lot of proteins floating around that can absorb the particles and raise a red flag to the immune system.
We want to have a particle stable for at least a couple of hours and then trigger drug release before the immune system recognizes it, or get these particles incorporated into the cells, where they’ll stay. But making a particle that will only release its drugs between two and eight hours, that’s a very difficult window to hit. That’s been the demise of many, many particles over the years, and we’ve had to deal with that in my lab.
How do you decide between biological or polymer-based particles?
I experiment with reverse engineering. So, based on what stimuli I want to take advantage of, my group and I think of what kind of material we can engineer, design, and fabricate.
I see what type of drug-release triggers I can apply based on where the cancer is in the body. If it’s melanoma, for instance, or something more superficial, then I can easily apply heat to that. And I can make phospholipids so they go through a melting process where they are a gel-like material at normal physiological temperatures, but when heated, they become much more fluid and leaky.
The other types, the plastic-type materials, have linkages within their structure. We can take advantage of acids present in the intercellular vesicles that attack and break down those linkages, so the particle falls apart and the drug is released.
What are your current challenges?
We use a range of chemotherapy drugs and we’re now experimenting with loading two different types of drugs into the same particle. If you’re a cancer patient, you’re usually not just receiving one chemo drug. It’s usually a cocktail of two to four different drugs, depending on the type of cancer.
Aside from chemo, I mentioned ultrasound that can heat tissue a few degrees or tens of degrees. Well, if you heat tens of degrees, then you’re destroying cells. We can use ultrasound heating to destroy cancer that isn’t responsive to other treatments. The concept has been around for decades, but it hasn’t been used because of a few limitations to the approach: it can take three to five hours to treat a patient, and the heating process must be monitored to prevent burning the skin or damaging healthy tissue.
Nathan McDannold and I have been using nanoparticles of liquid perfluorocarbon, which is already approved as an ultrasound contrast agent, so these droplets can collect in tumors and be vaporized with heat in gas bubbles that boost tissue absorption. The result is that you dramatically cut the energy needed, reducing the risk of burns, and you accelerate heating. We’ve reduced the time for killing a tumor by 50 percent.
What about your research on diseases besides cancer?
Our lab also works on gene therapy, and one of our targets is cardiovascular disease. If you remember, there was a lot of publicity and hubbub about gene therapy in the late 1990s once the genome puzzle had been figured out. In many cases, we know which gene potentially was mutated and we can reinsert that gene so the body makes a protein that was lacking or blocks a protein that is critical to the progression of a disease.
The big threat of cardiovascular disease is heart attack from a blockage in the coronary artery, which is actually due to inflammation. Say you have chronic inflammation in your arteries—certain proteins will be expressed in response to that inflammation, producing these clogging plaques. So we’re working on a way to reduce the expression of those pro-inflammatory markers. If we can do that, then we can mitigate the formation of these plaques.
The idea has merit and it’s been shown to work many times in the lab. The problem is delivery. Getting it to work in people is much harder. The genes are these little strips of genetic code. If we just inject them, the body has enzymes to break them down, and they won’t last more than a couple of minutes in circulation. It’s considered a harsh environment. So my group is developing nanomaterials to package and protect these sequences until they get where they need to go. Then we’re taking advantage of enzyme degradation or using ultrasound to trigger release locally.
How long before these therapies are available to patients?
There’s a messiness to the process of going from the lab bench to the patient’s bedside. We know that the ultrasound-triggered drug release works well, and we’re confident in the particle itself, but we have to get approval for the particle and approval for ultrasound to be used in this particular manner. Then there are sloughs of clinical trials; phase three is the big one, because what you’re proposing is to transform how patients are treated. You have to go up against the gold standard—what is currently used for patient care. Phase three trials are usually multicenter, involving hundreds or thousands of patients. It takes a whole lot of money. It’s not something a single researcher can sponsor; these are things that drug companies invest in heavily to get their product to market. So I’d say these medicines we’re working on are probably a decade out. There’s a lot more work that needs to be done.